Following on from Dr Jonathan Bujak’s first blog which documented the oceanographic and climatic changes that occurred in the North Atlantic region during the Paleogene, this blog discusses their application for the analysis of UK and Norwegian wells in energy exploration and carbon capture & storage.
Middle to Late Paleocene uplift of the Greenland Mantle Plume separated the North Sea and Barents marine systems from a North Atlantic Ocean that included the Rockall Trough and eastern Canadian offshore basins. The North Sea system, discussed in this blog, extended from the North Sea to the Norwegian-Greenland Sea. At this time, the Faroe-Shetland Basin formed a relatively narrow NE-SW seaway separated from the Rockall Trough by the Wyville-Thomson Ridge (Figure 1).
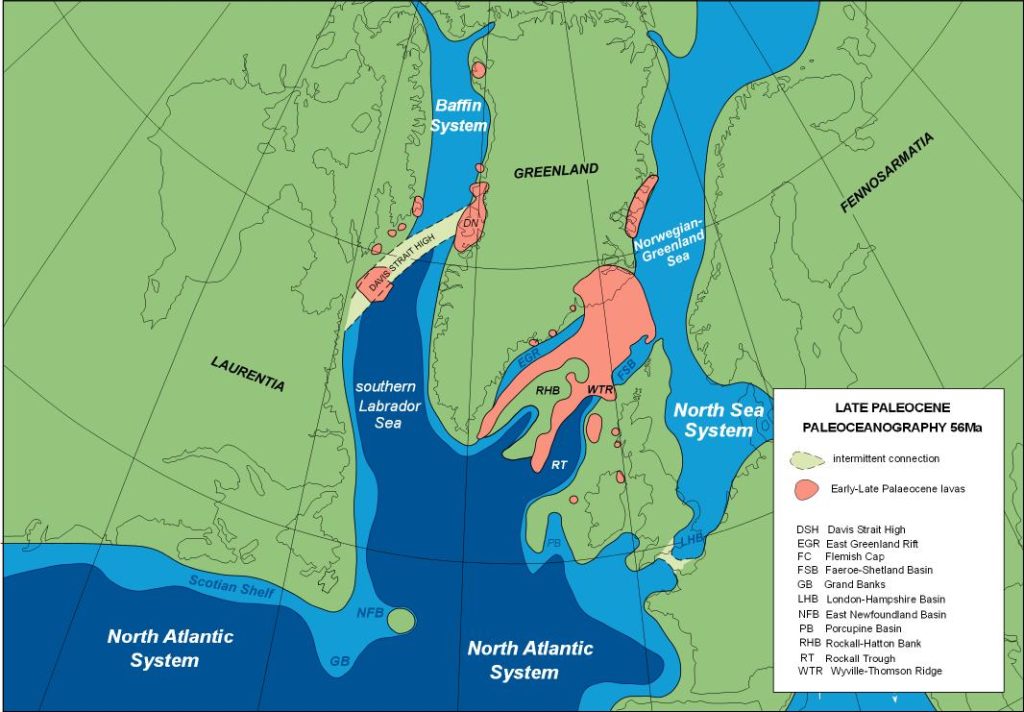
Warm greenhouse temperatures were punctuated by a succession of Middle-Late Paleocene hyperthermal events that approximately correlate with observed sequence boundaries (Figure 2). The hyperthermal events reflect pulses of uplift, basalt extrusion and emissions of greenhouse gases (GHG). High air and sea-surface temperatures during these hyperthermal events increased the hydrological cycle including evaporation and precipitation. This fuelled river discharge and therefore increased transportation of sediments and organic nutrients from the emerging land into basins. This, combined with lower oxygen solubility in the warm water, resulted in the development of plankton blooms, dead zones and deposition of organic material, including plankton-sourced sapropel.
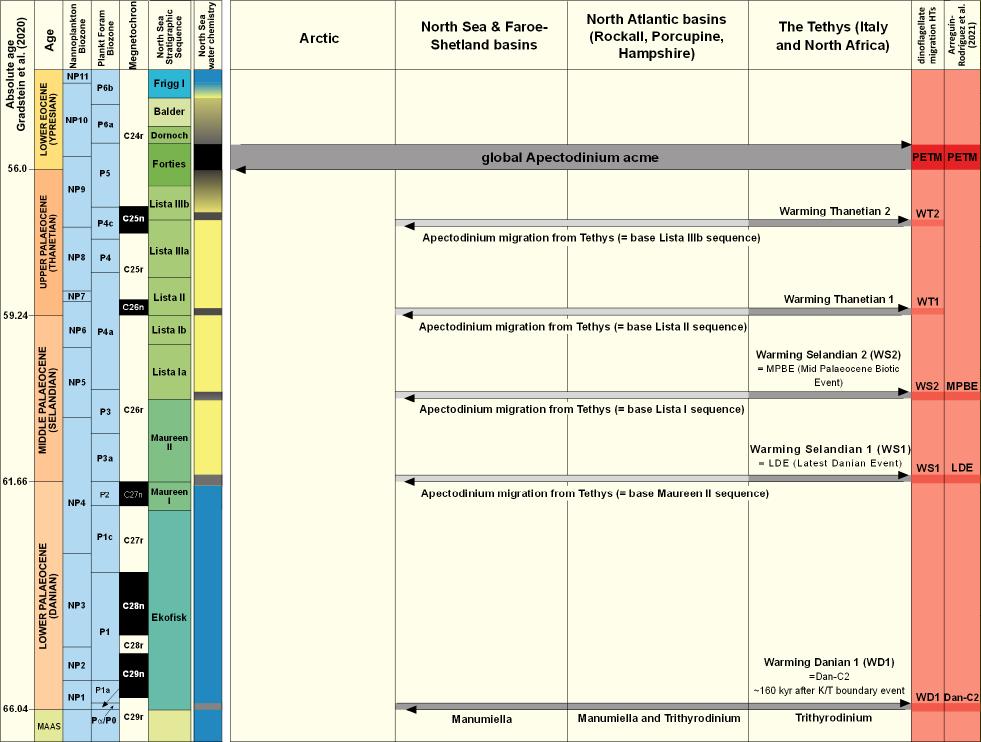
Occurrences of potential source rocks depend on favourable sedimentary, tectonic and geothermal conditions within individual basins. The younger sections reflect progressive plume uplift which led to basin enclosure, marine restriction and stronger hyperthermal events, as basalt extrusion and GHG emissions increased. The highest potential for source rock deposition was during the Paleocene-Eocene Thermal Maximum (PETM).
Peak uplift and igneous extrusion occurred during the ‘North Sea Biotic Crisis’ which had a massive effect on the benthos and plankton of the Forties, Dornoch and Balder sequences. Foraminifera were progressively eliminated and replaced by low-diversity coscinodiscid diatoms. Palynomorphs including thermophilic pollen and dinoflagellates including Apectodinium migrated globally during the PETM (Figure 3).

The combined succession of temperature-sensitive marine and non-marine dinoflagellate and pollen events enables correlation of sections deposited across marine, paralic and non-marine settings including those in the Faroe-Shetland Basin (Figure 4).

The Faroe-Shetland Basin contains the Rosebank oil and gas field which, according to Equinor, will be able to produce up to 8% of Britain’s oil leading up to 2030, augmenting the UK’s domestic energy needs through the energy transition. Rosebank ‘lies mainly within UKCS Block 213/27a, with extensions into Blocks 213/26b, 205/1a and 205/2a’ (Duncan et al. 2020). The (mostly) non-marine Colsay reservoir sands (Upper Paleocene-Lower Eocene) comprise braided bars and shales interbedded with lavas of the Lower Flett volcanics deposited during the Biotic Crisis (Figure 2 in Duncan et al 2020).
Biostratigraphic correlation and reconstruction of the events leading to the Biotic Crisis and PETM in the region of the field will help maximise Rosebank’s potential, and help identify the occurrence of similar fields.
The Paleocene-Eocene Thermal Maximum
The analysis of 1155 North Sea and Faroe-Shetland wells within the Bujak well database (available for purchase here) reveals a clear relationship between pulses of plume uplift, igneous activity, GHG emissions and Middle Paleocene to earliest Eocene hyperthermal events. Sediments deposited during the hyperthermal events contain Apectodinium that migrated from lower latitudes. This distinctive carnivorous dinoflagellate increased in numbers and diversity as the hyperthermal events intensified, culminating in the PETM (Bujak & Brinkhuis 1998).
Data from a coeval hydrothermal vent beneath the Norwegian-Greenland Sea provides a record of events that occurred during the PETM, including a large and rapid initial pulse of methane (CH4) release followed by up to 12 more pulses. Frieling et al. (2016) calculated that 1,500 billion tonnes of carbon were released from submarine vents during the PETM, concluding that ‘CH4 from the Norwegian Sea vent complexes was likely the main source of carbon during the PETM’.
Methane is a potent greenhouse gas that is mostly oxidised in the atmosphere to CO2 and water within 8 to 12 years, but it can remain in the stratosphere for ~120 years. On a 100-year timescale it has 28 times greater Global Warming Potential than CO2 and is 84 times greater on a 20-year timescale (European Commission 2023). (Global Warming Potential is a measure of how much infrared thermal radiation a greenhouse gas will absorb relative to CO2). It is therefore probable that some of the earlier Paleocene hyperthermal events resulted from a combination of Greenhouse Gases emitted by volcanic events that triggered the release of methane from submarine clathrates, with the intensity increasing in the younger hyperthermal events.
Hyperthermal and uplift events are often marked by the presence of coeval basal sands as illustrated in Figure 5 showing the 208/19-1 well of the Faroe-Shetland Basin. This also explains why hyperthermal events often correlate with sequence and lithostratigraphic boundaries.

208/19-1 shows the following relationship of hyperthermal events with uplift events and sands following Warming Danian 1 (WD1) that resulted from the end-Cretaceous bolide impact:
- WS1 associated with regional uplift at the base of the Vaila Formation and Maureen II sequence.
- WS2 associated with uplift and deposition of Vaila 3 sands at the base of the Lista Ia sequence.
- WT1 associated with regional uplift and Kettla tuff at the base of the Lamba Formation and Lista II sequence.
- WT2 associated with uplift and deposition of Lamba 3 sands at the base of the Lista IIIb sequence within the Lamba Formation.
- PETM associated with major regional uplift and deposition of Colsay sands at the base of the Flett Formation and Forties sequence.

Correlating nine Fareoe-Shetland wells (Figure 6) shows the relationship of hyperthermals to sands and volcanics within the basin. The 208/15-1 well, furthest NE on the section, has little sand development but shows the relationship between Thanetian WT1 and the Kettla tuff at the base of the Lista II sequence. Wells to the northeast, including 208/19-1, have thinner Middle to Upper Paleocene sections which thicken to the southwest. Quadrant 214 wells (214/27-1, 214/27-2 and 214/28-1) contain well-developed Vaila and Lamba (Paleocene) sands with Early Eocene Upper and Lower Colsay sands developed in 214/28-1.
Kettla tuffs and their igneous equivalents occur across the section at the base of the Upper Paleocene section, coincident with mid Paleocene regional uplift and WT1. The relationship between hyperthermal events, uplift events and initial sand deposition is clearly shown in 214/27-2.
Moving further southwest, Early to Middle Paleocene Vaila sands are absent or thin in Quadrant 206 wells (206/1-2 and 206/2-1A). However, Upper Colsay and Hildasay shelf sands are well developed. These intergrade into non-marine and shelf sands in 205/9-1 that include a succession of Colsay sands (Colsay 1 to 4) separated by Forties lavas in the 205/9-2 discovery well and illustrated for the 213/27-1Z type well by Duncan et al. (2020).
By using temperature-sensitive pollen and dinoflagellate in conjunction with other occurrence and abundance events, key reservoir sections deposited during, and immediately prior to the Biotic Crisis can be correlated in non-marine, paralic and marine environments. Their integration reflects changing air and sea-surface temperatures irrespective of local environments across the North Atlantic region including the North Sea, Faroe-Shetland Basin and Norwegian-Greenland Sea, discussed in detail in Bujak (2023). A similar approach was taken by Schofield & Jolley (2013) who used ‘Local Assemblage Zones’ to correlate the Colsay and Hildasay sands, using 205/9-1 as an example of the ‘assemblage biostratigraphy’.
This blog is based on Dr Jonathan Bujak’s synthesis of more 50 years’ work including more than 1000 NE Atlantic, 350 NW Atlantic (eastern Canadian) and 400 Arctic wells. His data and interpretations of 1155 North Sea and Faroe-Shetland wells are available from Merlin Energy Resources and Dr Jonathan Bujak based on his analyses of key reference wells and interpretations of all wells in 2020-2023.
References
Bujak (2023). Paleocene-Eocene high-resolution stratigraphy, paleoceanography and paleoclimate of the North Sea and Faroe-Shetland Basin. Non-exclusive report available from Dr Jonathan Bujak (jonathanbujak@outlook.com).
Bujak & Bujak 2020. The Azolla Story. Published by The Azolla Foundation as an e-book and print. Available from Amazon.
Bujak JP & Brinkhuis H. 1998. Global warming and dinocyst changes across the Paleocene/Eocene boundary. In: Paleocene/Eocene Boundary Events (Lucas, S.G., Berggren, W. & Aubry, M.-P., eds), Columbia University Press, pp. 277-295.
Bujak JP & Mudge DC. 1994. A high-resolution North Sea Eocene dinocyst zonation. Journal of the Geological Society of London, vol. 151, pp. 449-462.
Duncan LJ et al. 2020. The Rosebank Field, Blocks 213/27a, 213/26b, 205/1a and 205/2a, UK Atlantic Margin. United Kingdom Oil and Gas Fields: 50th Anniversary Commemorative Volume, G Goffey, JG Gluyas (eds). Geological Society London Memoirs 52(1), pp. 980-989. DOI: 10.1144/M52-2018-42
Gradstein FM, et al. 2020. Geologic time scale 2020, 2nd Edition, 1 March 2021. Amsterdam: Elsevier.
Mudge DC & Bujak JP. 1996. An integrated stratigraphy for the Paleocene and Eocene of the North Sea. In: Correlation of the Early Paleogene in Northwest Europe. (Eds Knox RWO’B et al.), Geological Society Special Publication No. 101.
Mudge DC & Bujak JP. 2001. Biostratigraphic evidence for evolving palaeoenvironments in the Lower Paleogene of the Faeroe-Shetland Basin. Marine and Petroleum Geology, vol. 18, pp. 577-590.
Schofield N & Jolley DW. Development of intra-basaltic lava-field drainage systems within the Faroe–Shetland Basin. Petroleum Geoscience, vol. 19, pp. 273 – 288.